Fusing the future of energy
Photo credit: © NASA/CXC/INAF/Argiroffi, C. et al./S. Wiessinger
Nuclear fusion was once seen as fantasy physics among many science commentators: “It’s thirty years away and always will be,”[1] used to be the familiar phrase. Yet there are strong signs that fusion energy, far from being a fantasy, may soon become a reality. And, in doing so, help to completely transform the world’s reliance on fossil fuels.
These days, media headlines such as “Nuclear fusion market could achieve a US$ 40 trillion valuation”[2] from Bloomberg Intelligence and “Nuclear fusion enters a new age”[3] in Forbes reflect a growing excitement that this power source might finally become viable for everyday use. So, what’s really changed to turn conventional opinion on its head? And, more importantly for the climate and energy crisis, just how soon could fusion energy become an accepted part of the global energy mix?
© Courtesy of Massachusetts Institute of Technology (MIT) and the MIT Plasma Science and Fusion Center.
Unfulfilled potential?
The advantages of fusion are significant – unfortunately so are the technological barriers. Progress over the past half century has been both slow and yet, encouraging.
In February 2022, researchers at the Joint European Torus (JET) facility operated by Culham Centre for Fusion Energy in Oxfordshire, UK, announced a new record for nuclear fusion after they successfully produced 59 megajoules of energy – enough to run a 60-watt light bulb for 11 days. Hardly game-changing in terms of the amount of energy produced, this was nevertheless hailed as a sign that fusion, a process promising limitless, clean energy, was inching towards becoming a reality. The previous record, also set by JET, was set 25 years earlier when it released 22 megajoules in 1997.
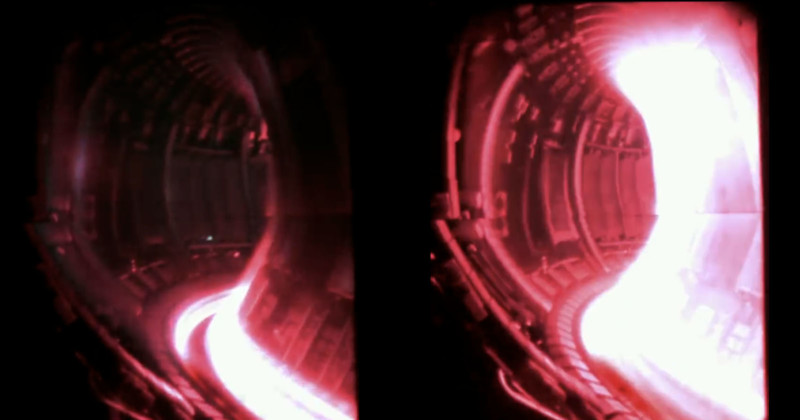
Meanwhile, the International Thermonuclear Experimental Reactor (ITER) being built in southeast France, has been under construction for 11 years and is tens of billions of dollars over its initial US$6 billion budget. Its current US$22 billion build cost is being met by the governments of two-thirds of the world’s population, including the EU, the US, China and Russia[4].
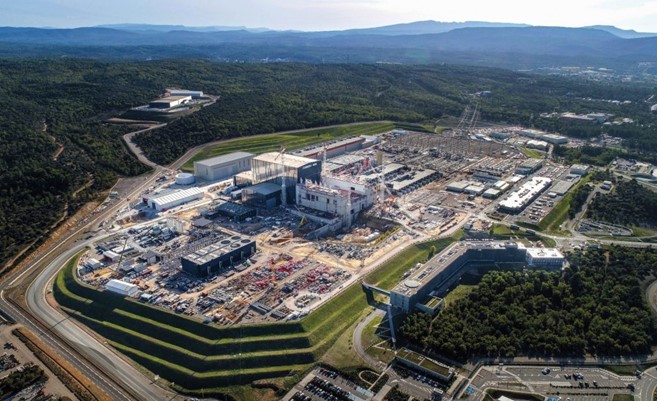
Of course, there are several other alternatives to fossil-fuel energy, including concentrated solar, solar photovoltaic power, on/off-shore wind farms, biomass and geothermal, to name a few, that are already making a major contribution to the energy transition necessary to achieve net zero. But the potential benefits of nuclear fusion are still attracting investment from the likes of Jeff Bezos[5], Bill Gates[6] and, indeed the Jameel Family’s investment arm, JIMCO, part of Abdul Latif Jameel’s longstanding commitment to sustainable forms of energy, such as wind energy and solar power.
Benefits of fusion energy
Carbon emissions, including greenhouse gases (GHG) such as carbon dioxide and methane, are a known key driver of planetary climate change. The more GHGs there are in the atmosphere, the warmer the climate becomes. And despite our best efforts, the situation is getting worse. In 2021, the global average carbon dioxide set a new record high of 414.72 parts per million[7].
The beauty of nuclear fusion is that there are no carbon emissions. And, unlike the more familiar energy from nuclear fission, fusion reactors produce no high activity, long-lived nuclear waste. The only radioactive activation is of the actual components of the fusion reactor itself and this is low enough for the materials to be recycled or reused is less than 100 years[8].
Unlike the fuels of nuclear fission – so-called ‘fissile materials’ – for example uranium or plutonium, the raw materials used in nuclear fusion are plentiful, harmless (neither a fissile, fissionable, not enriched in anyway), meaning there is no risk of weaponization, and they are significantly easier to obtain. Deuterium can be extracted from water and tritium will be produced inside the power station from lithium, an element that is abundant in the earth’s crust and seawater.
The process is also very productive: one kilogram of fusion fuel could provide the same amount of energy as 10 million kilograms of fossil fuel. A one-Gigawatt fusion power station would consume less than one ton of fuel during a year’s operation. A single gram of fuel can yield 90,000-kilowatt hours of energy. Safety is also a major consideration. The history of conventional nuclear fission power is littered with accidents and near misses. Authorities worry about the risk of key components falling into the wrong hands and being used to build nuclear weapons. Not so with fusion energy. As noted above, the amounts of fuel used are miniscule – the weight of a postage stamp at any one time – and not ‘fissible’ or enriched – so there is no chance of a meltdown or runaway reaction.
“I like to say to tell people that nuclear fission is easy to start and hard to stop. Fusion energy is the opposite,” explains Christofer Mowry, former Chief Executive Officer of one of the major players in the sector, Canada’s General Fusion[9].
Advocates of fusion power plants says they have the potential to produce an almost limitless supply of energy, at low cost and with minimal risk[10] – benefits that are truly worth pursuing.
Fusion vs. Fission
Conventional nuclear power stations rely on fission, i.e., the splitting of heavy element atoms, usually uranium, into lighter ones. The energy that’s released is used to boil water to create steam, which then turns turbines that make electricity.
Fusion is the opposite process. It generates heat by combining (or fusing) lighter atoms to make heavier ones. The reason fusion generates so much energy is that the new element weighs ever so slightly less than the sum of its parts. That tiny bit of lost matter is converted into energy according to Albert Einstein’s famous formula, E = mc2. This is the same process that generates the power of the Sun and other stars. In Einstein’s formula, “E” stands for energy and “m” for mass. The last part of the formula is “c,” a constant that measures the speed of light — 300,000 kilometers per second. This is then squared – an enormous multiplier for matter that is converted into energy, making fusion an extraordinarily powerful reaction[11].
However, this is not a simple process. For fusion to work, atoms must collide. The problem (or one of them) is that their nuclei – the positively charged cores of atoms, inside a negatively charged electron cloud – normally repel each other. To make overcome this repulsion force, the atoms need to be moving incredibly fast in a confined space. Our Sun, and other stars, can do this because their, literally, astronomical mass generates enormous gravitational forces that makes atoms hurtle towards their center.
Here on Earth, we need a different approach. This is what has been preoccupying our finest physicists and engineers for decades. The fusion process has been demonstrated at small scale in laboratories, but such is the complexity of the process, it has yet to generate more energy than it consumes.
The reaction requires the creation of a high-energy state of matter: plasma. This is an ionized state of matter similar to a gas. Composed of charged particles (positive nuclei and negative electrons), plasmas are very tenuous environments, nearly one million times less dense than the air we breathe[12]. Plasma requires very high temperatures – 50 million degrees Celsius – and must be kept stable under intense pressure, and dense enough and confined for long enough to allow the nuclei to fuse.
Differing approaches to fusion
For fusion to be useful, plasma must be created in a controlled way so the resulting energy can be harvested. There are currently two fundamental approaches, with some variations. One, adopted by the National Ignition Facility at Lawrence Livermore National Laboratory in California, is to confine the fusion fuel and compress it using lasers. This is called inertial-confinement fusion (ICF).
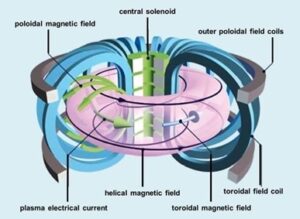
Illustration © courtesy of EUROfusion / US Dept. of Energy.
The other approach is generally referred to as magnetic-confinement fusion (MCF), and employs extremely powerful magnets that create a magnetic field – a ‘bottle’ – to insulate and contain the plasma. At the heart of this is a doughnut-shaped type of MCF reactor called a tokamak. This uses high-intensity magnetic fields to heat a hydrogen plasma to hundreds of millions of degrees Celsius, and then hold that plasma stable while its atoms combine. The goal is to hold the plasma together long enough for a significant amount of fusion to occur. The current record is just six minutes.
A variation on these approaches is Magnetized Target Fusion (MTF), which combines features of both MCF and ICF, but requires less engineering and fewer resources. Like the magnetic approach, the fusion fuel is confined at lower density by strong magnetic fields while it is heated into a plasma. The fusion reaction is initiated by rapidly squeezing the target to greatly increase fuel density and temperature as in the inertial approach. The resulting density is lower than in ‘pure’ ICF, and some scientists believe this hybrid approach, offering extended confinement time and improved heat retention, will ultimately enable MTF and be easier to construct.
The belief is that a fully-fledged commercial fusion reactor will be viable enough to compete with other forms of electricity. The target is a cost of US$ 50 per megawatt-hour, which is the same as coal. Although other forms of renewable energy might be cheaper, they are not as reliable or as flexible.
Investing in tomorrow’s energy
As a committed investor in sustainable energy and the drive to net zero, Abdul Latif Jameel – through the Jameel Investment Management Company (JIMCO) – has chosen to invest in two of the global pioneers in the rapidly developing fusion energy sector: Commonwealth Fusion Systems (CFS) – an MIT Plasma Science and Fusion Center spinout based in Boston that counts Jeff Bezos and Bill Gates among its backers[13] – and Canada-based General Fusion, which is also backed by Bezos.
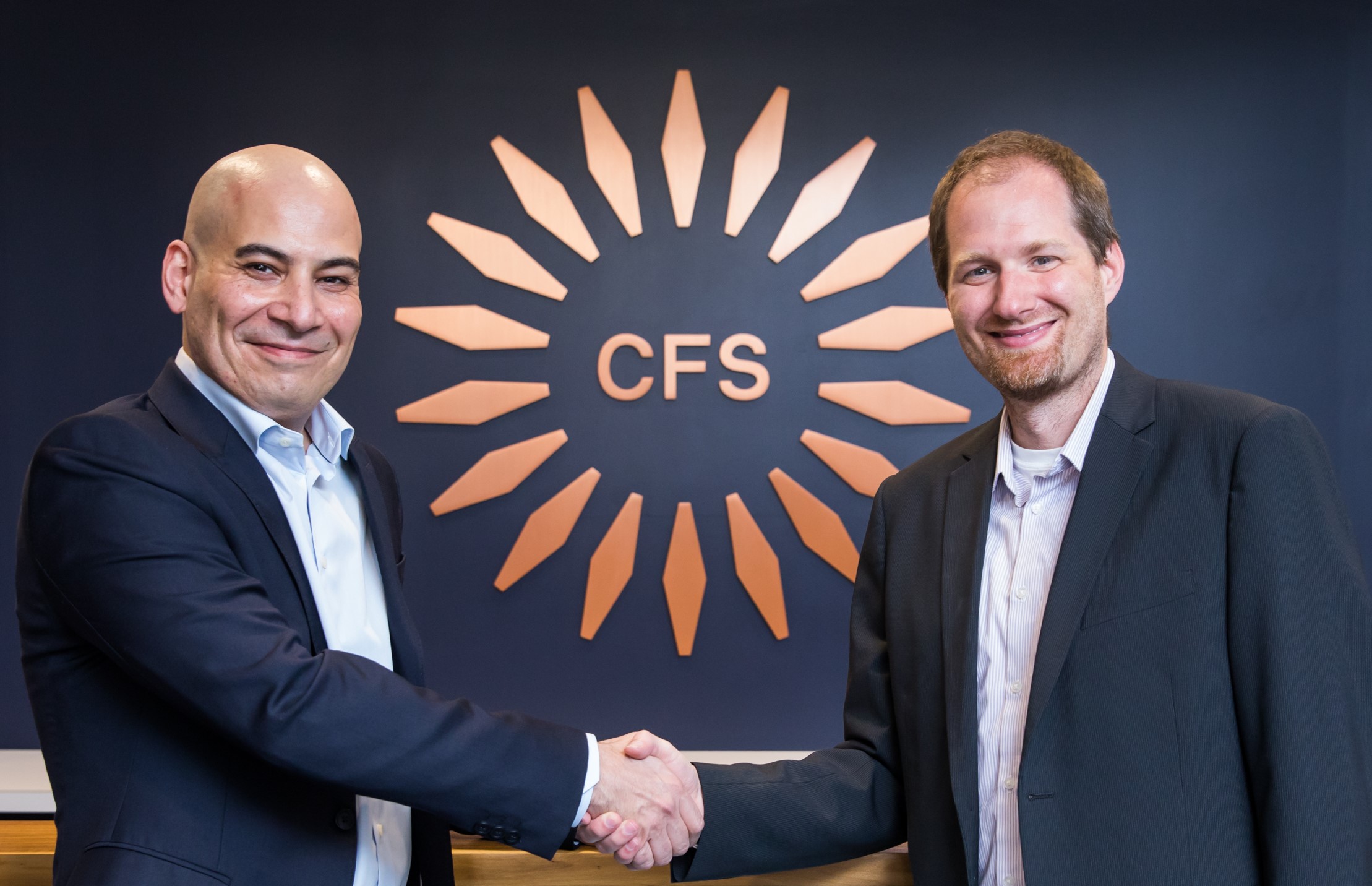
In December 2021, the JIMCO Technology Fund participated in CFS’s US$ 1.8 billion Series B funding round to accelerate the commercialization of fusion energy. That same month, it also announced its participation in General Fusion’s Series E financing round of US$ 130 million.
General Fusion’s technology uses the MTF approach outlined above to plough a middle furrow between MCF and ICF. In this process, the reactor avoids the need for magnetic confinement by using powerful electric pulses to create self-stabilizing puffs of plasma that are then injected into the reactor’s core. It has been likened to a smoke ring, in which the air currents within the ring maintain its shape for a few seconds before it vanishes[14]. The puffs of plasma only last around 20 milliseconds, but that is long enough for them to be compressed.
The core of General Fusion’s reactor will be lined with molten lithium and lead. Once a puff of plasma has been injected, ranks of gas-driven pistons will compress the core, changing it from a cylinder to a sphere and drastically boosting the fusion rate.
The reactor’s liquid-metal jacket not only compresses the plasma but also captures the energy from the reaction. Heated metal will be piped to a heat exchanger and used to raise steam. Neutrons from the fusion reaction, meanwhile, will transform some of the lithium into more tritium fuel, which would otherwise be rare and expensive. The reactor can also increase or decrease power output ten-fold by changing the speed at which the core cycles. This should allow it to ‘load-follow’, ramping up production when electricity demand is high and cutting back when it is low.
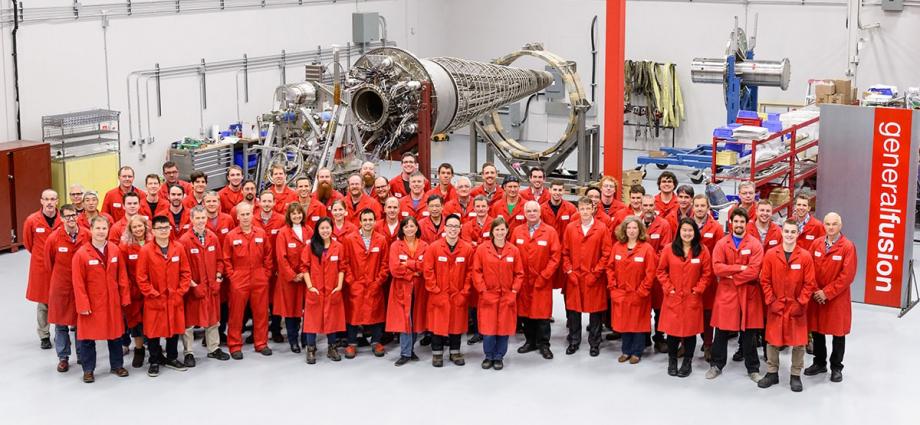
Laser compression, such as that used at the National Ignition Facility, takes billionths of a second. But compression in the General Fusion reactor takes thousandths of seconds, bringing it within the range of far cheaper digital electronics. The result should be a reactor that is cheaper and simpler to build and operate than either an MCF or an ICF machine.
General Fusion is constructing a demonstration plant at Culham, near London – the hub of UK fusion research for decades – that is scheduled to start operating in 2025. The company aims to have its first reactors on the market in the early 2030s.
Taking on the tokamak
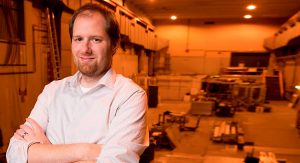
Boston-based Commonwealth Fusion Systems is taking a different approach, based on tokamak technology. Bob Mumgaard, Chief Executive Officer and Co-founder, CFS, says the company aims to have a working reactor in six years.
In a recent interview for an Abdul Latif Jameel Perspectives article, Mumgaard explained how his company used ground-breaking high-temperature superconductors (HTS) made from rare earth barium copper oxide to make powerful magnets far smaller than ever before.
This means that the tokamak used in the reactor can also be far smaller.
“Current tokamak technology requires them to be very large to create the magnetic field to achieve net energy for fusion,” he said, pointing out that the site for the ITER plant in France is 1 kilometer long and 400m wide. “By developing these HTS magnets in the right configurations, we can now make much, much smaller tokamaks – about 40 times smaller than ITER, for example – which is a game-changer for fusion systems.”
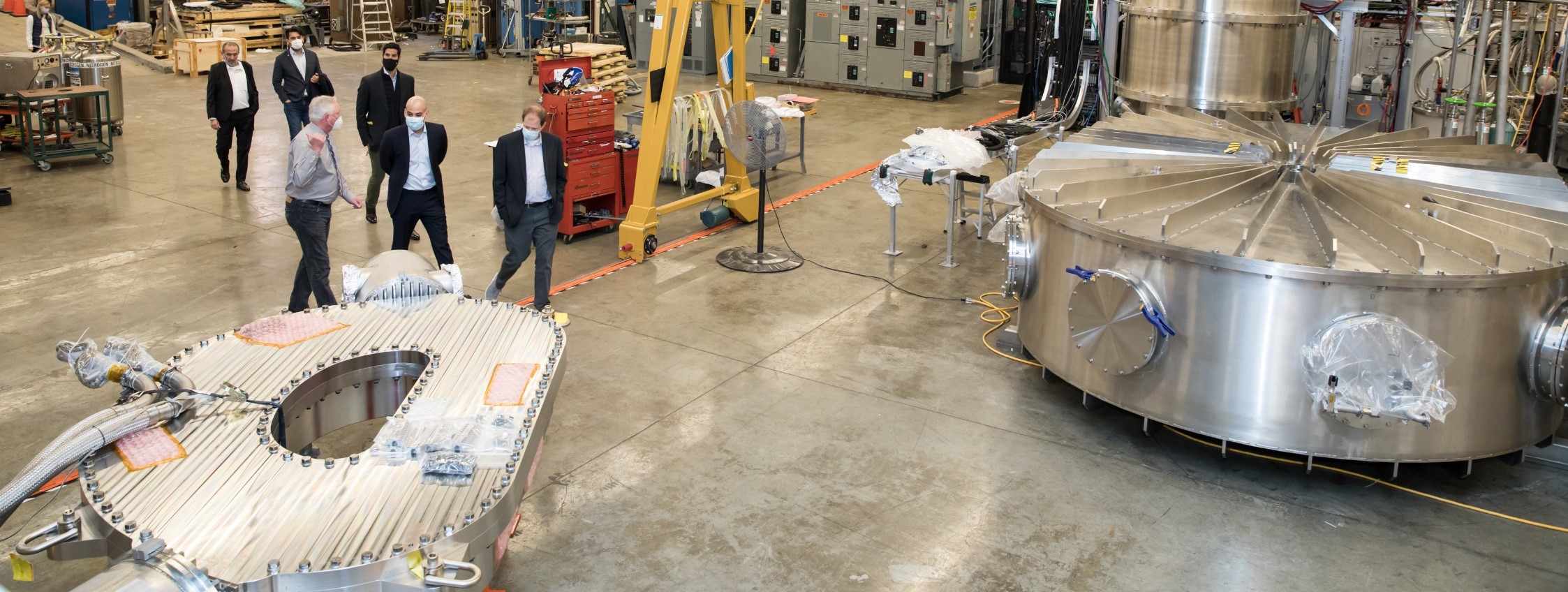
To give an idea of the strength of the HTS magnets, the power of magnets – the magnetic field strength – is measured in a unit called Teslas.[15] The magnets used by CFS in its reactors are 20 Teslas – big enough to lift a battleship. Mumgaard calls the tokamak a “big magnetic bottle” where powerful magnetic fields control balls of 100-million-degree plasma — “star stuff.”
Having successfully the proof of concept in September 2021, MIT and CFS together are constructing what Mumgaard calls “the first fusion machine that makes net energy” — that is, the first fusion plant that will produce more energy than it consumes; 10 times more energy, according to Mumgaard, with an output of 100 MW of power[16]. Named SPARC, it is being constructed in Devens, Massachusetts and is scheduled to be in operation by the end of 2025.
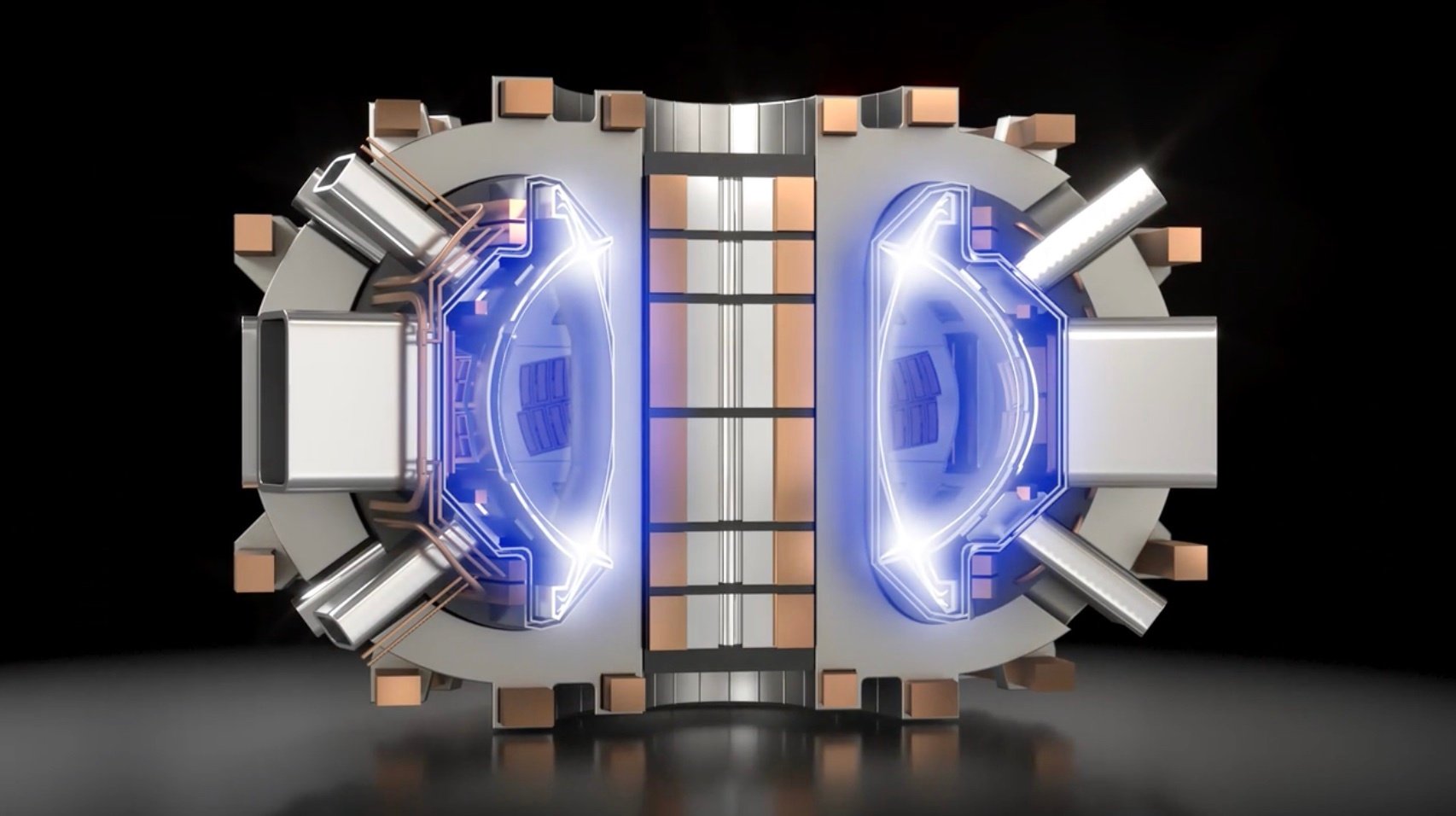
Power of private capital
Such are the huge sums of investment required to advance fusion technology, and the long payback horizons, that Fady Jameel, Deputy President and Vice Chairman of Abdul Latif Jameel, believes private investors are vital to the long-term success of fusion energy
“Private capital and family businesses both have the advantage of patience. They can afford to invest for the longer term because they don’t have a raft of shareholders demanding returns in three to five years,” he says. “As such, I believe they have a unique opportunity, arguably a responsibility, to invest in these types of breakthrough future technologies as part of their business continuity, and also as a responsibility for future generations.”
Abdul Latif Jameel itself has a long track record of investing in sustainable energy technologies. It also has its own businesses working in the renewables sector, such as Almar Water Solutions, a leading company in the development and management of water infrastructure and services, and Fotowatio Renewable Ventures (FRV), both part of Abdul Latif Jameel Energy. FRV has a range of projects developing sustainable energy, from its nine solar farms in Australia to Clay Tye, the UK’s largest battery storage development project, which will contribute to the decarbonization of Britain’s energy grid.
Rich rewards
With investment in fusion energy at record levels, driven largely by private capital rather than just government backing, there is a question: what is it worth?
Bloomberg Intelligence[17] believes that success – a commercially viable reactor – is so near that it is worth attempting an answer. Its paper on the subject says commercially viable fusion energy would be an achievement “off the Richter scale of modern advancements”. It believes Tesla (the motor manufacturer) is the closest comparator, even though the consequences of fusion energy are far greater.
Assuming that fusion could replace 1% of the world’s energy gigawatt output, this would imply a US$ 40 trillion valuation, it concludes. Far more than that, fusion energy would turn the energy market on its head and, in the process, transform what we thought possible. Limitless, clean, renewable and affordable energy for all.
Surely a future worth investing in?
[1] https://www.economist.com/science-and-technology/2021/06/24/the-race-to-build-a-commercial-fusion-reactor-hots-up
[2] https://www.bloomberg.com/professional/blog/nuclear-fusion-market-could-achieve-a-40-trillion-valuation/
[3] https://www.forbes.com/sites/christopherhelman/2022/01/02/fueled-by-billionaire-dollars-nuclear-fusion-enters-a-new-age/
[4] https://www.sciencefocus.com/future-technology/fusion-power-future/
[5] https://www.cnbc.com/2019/03/06/bezos-microsoft-bet-on-a-10-trillion-energy-fix-for-the-planet.html
[6] https://www.nasdaq.com/articles/bill-gates-and-big-oil-are-chasing-the-nuclear-fusion-dream-2020-06-03
[7] https://www.climate.gov/news-features/understanding-climate/climate-change-atmospheric-carbon-dioxide
[8] https://www.iter.org/sci/Fusion
[9] https://www.economist.com/science-and-technology/2021/06/24/the-race-to-build-a-commercial-fusion-reactor-hots-up
[10] https://ccfe.ukaea.uk/fusion-energy/fusion-in-brief/
[11] https://www.vox.com/22801265/fusion-energy-electricity-power-climate-change-research-iter
[12] https://www.iter.org/sci/MakingitWork
[13] https://www.economist.com/the-economist-explains/2022/02/09/what-is-nuclear-fusion
[14] https://www.economist.com/science-and-technology/2021/06/24/the-race-to-build-a-commercial-fusion-reactor-hots-up
[15] The Tesla (T) is a derived unit of the magnetic B-field strength in the International System of Units. One tesla is equal to one weber per square meter. 1 T in: is equal to SI base units: 1 kg⋅s−2⋅A−1 Symbol: T Derivation: 1 T = 1 Wb/m2
[16] https://www.nature.com/immersive/d41586-021-03401-w/index.html
[17] https://www.bloomberg.com/professional/blog/nuclear-fusion-market-could-achieve-a-40-trillion-valuation/